Our special guest post this week comes from Dr. Liz Clark of Yale University in New Haven, Connecticut, USA. She continues to bring biomechanics-fu to echinoderms– the weird marine critters like seastars and sea urchins. Including fossils, as you’ll see today! You may remember her from blog posts such as “Guest Post: Brittle Star Arms Are Weird“.
Stomach-Churning Rating: 1/10; echinoderms are inoffensive.
Imagine that you’re stuck in a cardboard box on the beach, holding a small stick. Could you use the stick to move yourself forward? What would you do? You could try digging into the sediment ahead of you to pull yourself along. You could try rowing side to side, as if you were in a rowboat. Or maybe it’s not possible and you’d give up, decide to stay put, and wave your stick in the air for help.
Believe it or not, this is a strange-but-important dilemma that some paleobiologists- like me!- have been wrestling with for generations. My research specialty is in the biomechanics of locomotion– how organisms use their bodies to get from one place to the next (through walking or swimming, for instance). We can learn a lot about an animal by studying their locomotion, such as why their body is shaped the way that it is, or what role they occupy in their ecosystem. Animal motion is a major inspiration for robotic design, and I work with engineers to apply the novel insights on animal locomotion from my research to create new kinds of devices.
Studying the biomechanics of motion in living organisms is (relatively) straightforward. We can use high-speed cameras, motion capture software, and 3D imaging tools to visualize and understand how organisms move in real-time, informing our inferences about how they perform certain tasks. Inferring locomotion in fossil organisms, on the other hand, is tricky since we can’t observe the organism’s behavior like we could if the organism were alive. Instead of being able to watch the organism move, we’re left with a snapshot of the animal frozen in place in a rock. We’re also missing a lot of physical information: locomotion in most animals requires soft tissue and hard skeletal structures, but typically with fossils, only the hard structures get preserved.
However, we can often garner some insights from living organisms to determine the locomotion strategies that fossil organisms use. Most organisms in the fossil record look at least somewhat similar to organisms alive today. If our fossil has four legs, for instance, we can study locomotion in living tetrapods (four-legged animals) to help us create a framework for deriving inferences about locomotion in our extinct tetrapod fossil animal. But for some really strange-looking animals- ones without obvious modern analogues- we’re not so lucky. For me, this is where the fun begins.
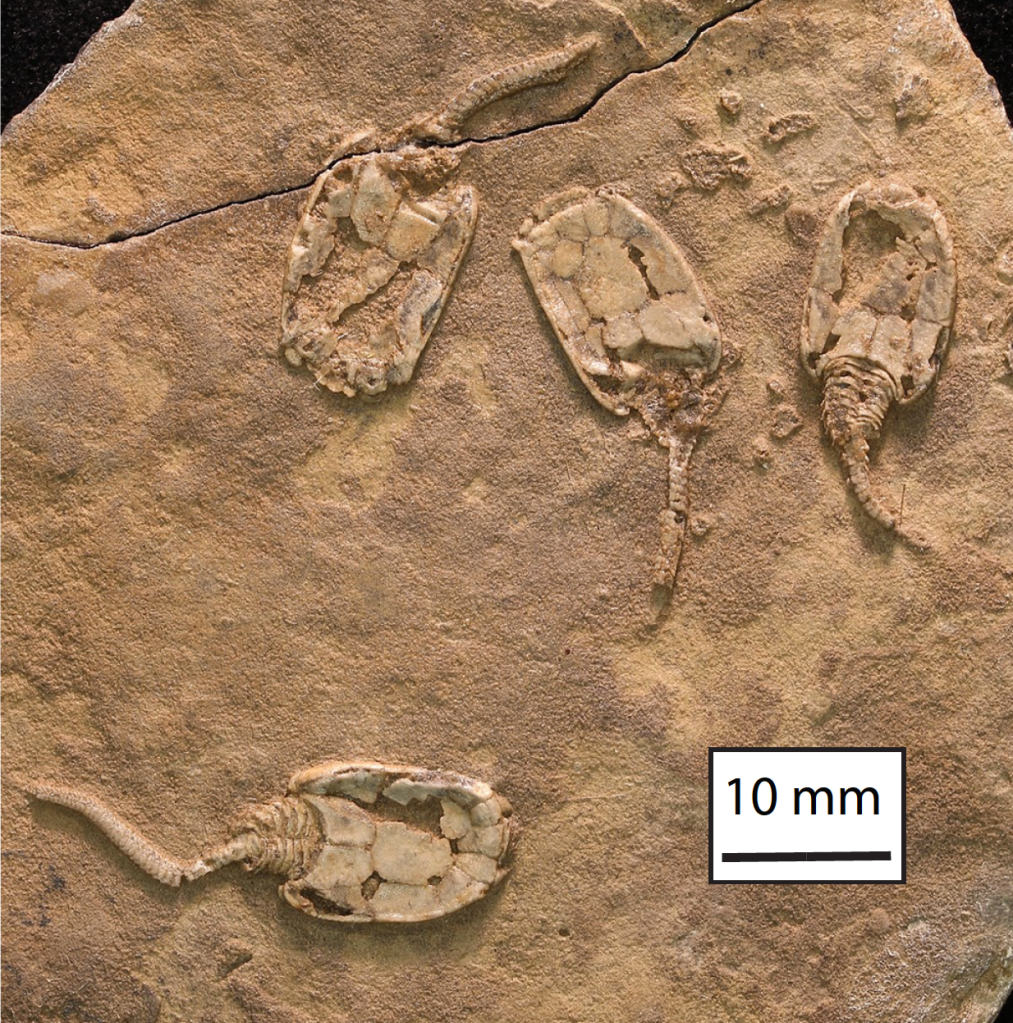
Figure 1: Stylophorans! Here are four fossilized stylophorans from the Helderberg Group of the Early Devonian (YPM 036413)
So getting back to the cardboard box and the stick. These are metaphorical examples of the different locomotion strategies that have been proposed for a group of fossil animals known as stylophorans (Figure 1). Stylophorans are extinct organisms related to sea stars and sea urchins, but with a body structure unlike any organism on the planet today. They have a large, relatively flat body called a theca (i.e., the cardboard box), and a long, thin segmented tail known as the aulacophore (i.e. the stick) (Figure 2). They’re known in the paleontological community as some of “the strangest-looking animals of all time.”
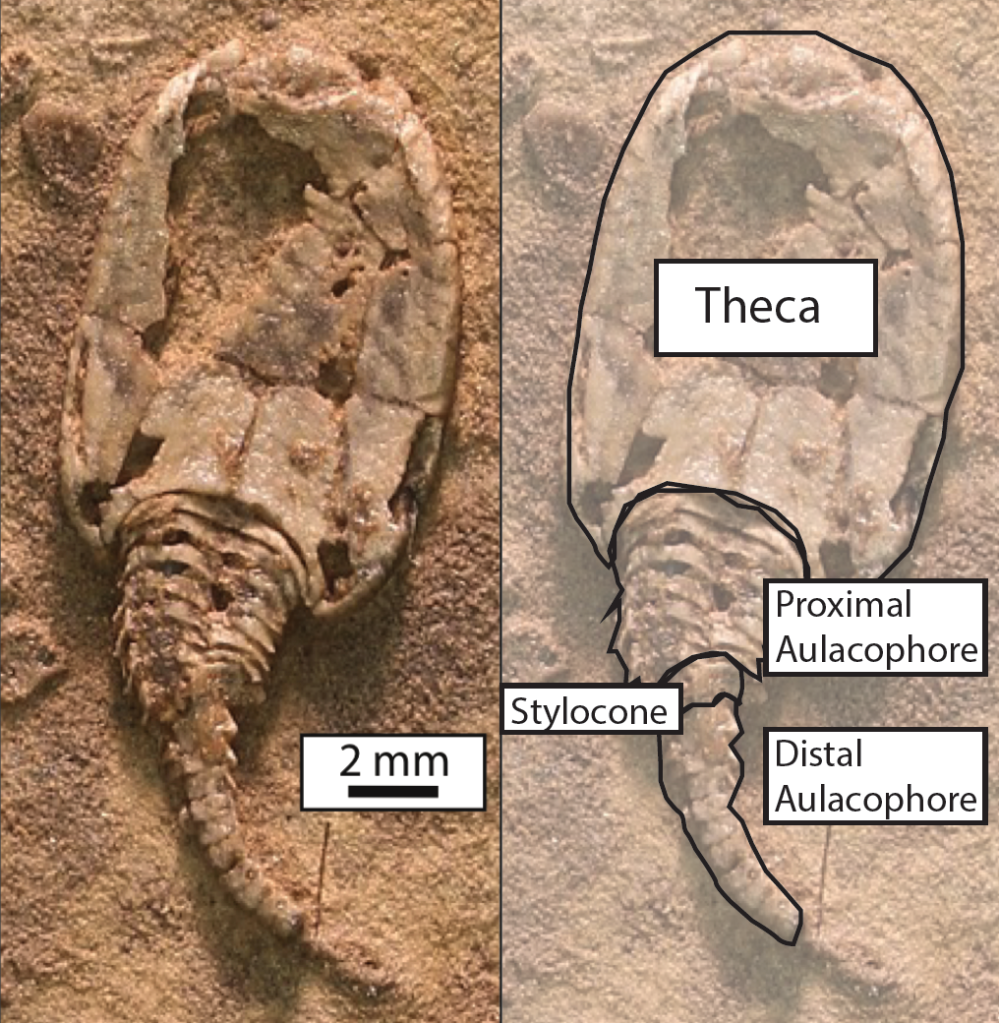
Figure 2: Stylophoran anatomy. The “theca” is the body cavity, and the “aulacophore” comprises of the proximal aulacophore, the stylocone, and the distal aulacophore.
By reconstructing stylophoran locomotion, we can unlock the mechanics of a unique system for motion and its potential applications to engineering. We can also understand more about how this organism lived and functioned in its ancient ecosystem. And, by developing a new approach to understand locomotion in stylophorans, we can apply this strategy to analyze locomotion and movement in other unusual fossil animals as well!
For years, scientists have been documenting the incredible array of stylophoran diversity in the fossil record and making their best predictions about how they would have been able to move (or not!). These predictions are based on their morphology– the structure of an organism’s body. For stylophorans, that means the shape and structure of the theca and aulacophore. There are a variety of stylophoran thecal shapes, ranging from ovoid in Enopleura to trapezoidal in Ceratocystis to almost crescent-shaped in Cortnurnocystis. There’s a similarly wide array of aulacophore morphologies as well.
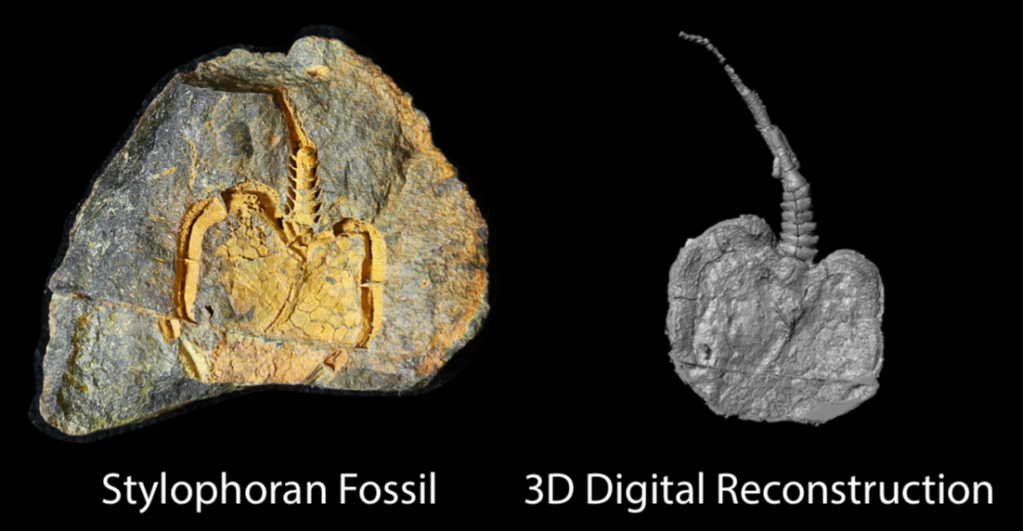
Figure 3: Left: One half of the concretion within which the stylophoran fossil we analyzed is preserved. Right: The 3D digital image of the stylophoran fossil, created by micro-CT scanning the fossil specimen.
We developed a new approach using 3D imaging (Figure 3) to create a digital model of a stylophoran specimen. We used the model to test if several different locomotion strategies that had been proposed before were physically possible or impossible for a stylophoran to actually perform.
First, we used a micro-CT scanner to image a fossil stylophoran. This outputs a digital 3D picture of the stylophoran fossil that we can look at and analyze on a computer. Next, we developed a program to calculate the joint centers- the point at which one skeletal structure rotates relative to another-within the digitized stylophoran’s aulacophore (Figure 4). This created a digital marionette– a rig of our stylophoran fossil that flexes at the junctures between aulacophore segments as it would have in life. We then rotated each segment at the joint center to calculate the aulacophore’s total range of motion– a reconstruction of how far the aulacophore could flex in each direction (Figure 5).
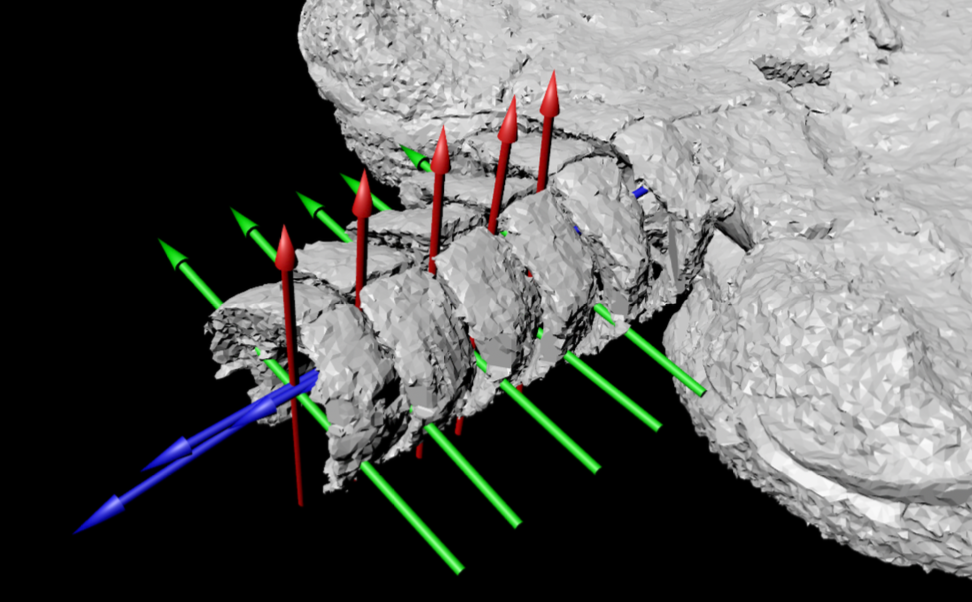
Figure 4: A look into some of the nuts and bolts of the 3D model we created. Tri-colored axes demarcate where the joint centers are in the proximal aulacophore.
We used this 3D range of motion model to evaluate several locomotion strategies that had been previously hypothesized for this group of stylophorans. One hypothesis suggested that these stylophorans dug their aulacophores into the substrate– sediment on the ocean floor- to pull themselves forward. Another suggested that they moved the aulacophore side to side in order to push themselves along. We found that the first hypothesis would have been impossible to conduct based on the range of motion we calculated, but the second strategy was theoretically possible! We’ll need to do more work to see how likely it was that stylophorans would have actually used this technique. Nevertheless, through this investigation, our team produced the first objective, data-driven methodology for analyzing locomotion in fossil invertebrates, which is a big step in the right direction for the study of fossil invertebrate biomechanics! Our technique can be applied to study other organisms with rigid skeletons as well, like crabs, insects, or sea stars, for instance, and we’re looking forward to seeing our technique used to uncover more interesting locomotion strategies!
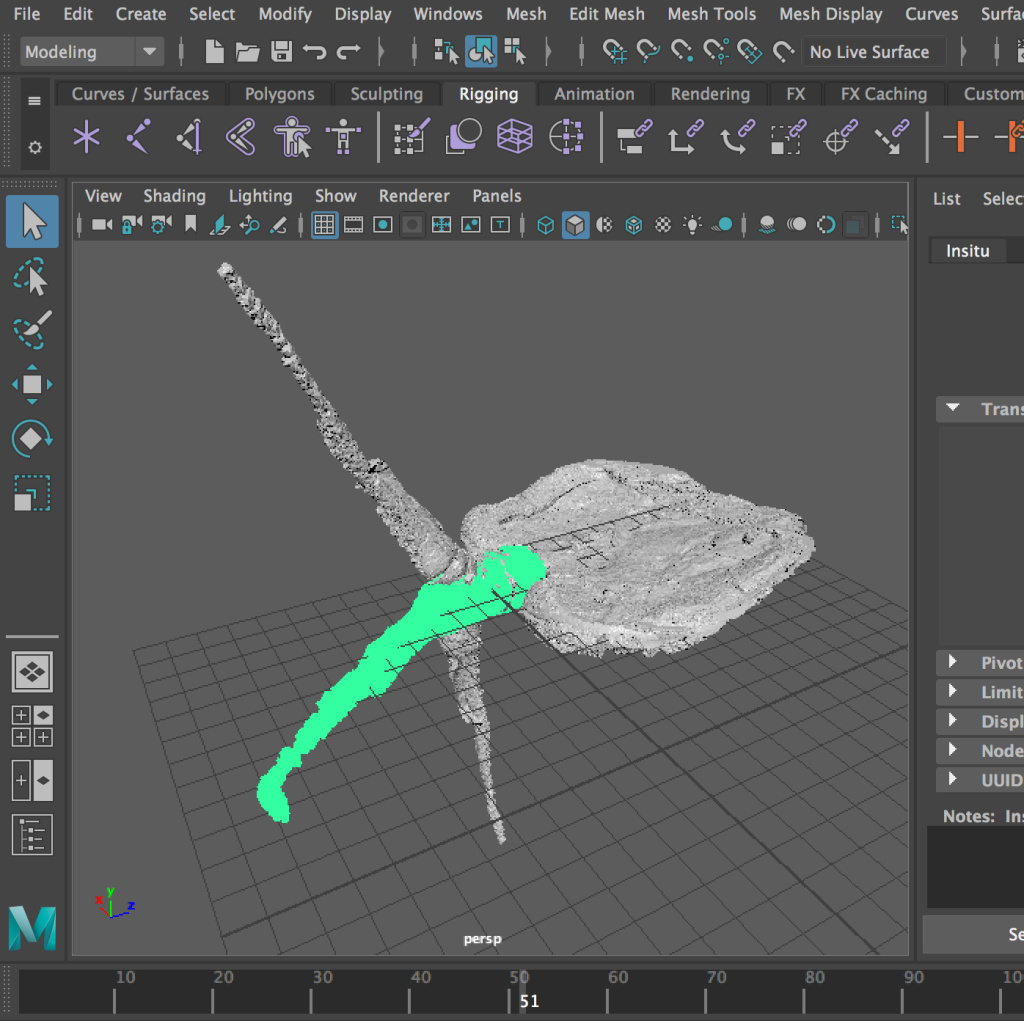
Figure 5: A snapshot of the 3D model where we can observe how dorsal and ventral range of motion compare to the originally preserved orientation of the aulacophore (highlighted in green).
Do you want to know more? You can! We published a paper on this topic here!